Fiber-based microphysiological systems: a powerful tool for high throughput drug screening
Introduction
There is an existing clinical need for reproducible disease models for improving drug and therapeutics screening rates. Evolved drug screening and testing methods on disease models can improve upon animal drug testing and traditional two-dimensional (2D) assays in both cost and efficiency (1). Fiber-based microphysiological disease models offer promising and realistic solutions to this clinical need, chiefly in physiological tissue types where cell alignment is important to the biological function, and tissues that are fibrous in nature. These constructs may be fabricated using different methods to model physiological tissues; various manufacturing and assembly methods for microphysiological fiber-based systems can demonstrate biomimicry of epithelial, connective, skeletal muscle, smooth muscle, and peripheral nervous tissues. These fiber-based constructs offer flexibility in fiber size, length, assembly of fibers, pore size, and material composition. Different fiber manufacturing methods allow for organic and synthetic extra-cellular matrices (2), fibers with complex surface morphologies (3), and multi-material coaxial fiber formation (4), for example. The material properties of these structures can be further manipulated by using textile assembly methods to create multifunctional systems.
A general decline in the productivity of pharmaceutical research indicates that drug screening processes need to be redesigned to more dependably recapitulate the pathological mechanisms of human disease, without assay, target, or compound biases (5). Animal model drug toxicology tests oftentimes provide limited data with questionable relevance to the human disease or condition; they allow for an understanding of molecular targets, but are expensive and lengthy to run (1). Since 1996, high throughput drug screening of 2D miniaturized cell-based assays has been a regulated process used in drug design for identifying compounds that cause undesired side effects in the target drug (6). However, 2D assays do not show the full picture of drug toxicology effects that are evident in an in vivo 3D model. The time and collateral limitations of current drug screening methods can be addressed by bridging the gap between in vitro and in vivo (1). These issues may potentially be overcome, and drug screening throughput increased, by fiber-based microphysiological systems which mimic the physiological environment of native tissues. Engineered tissues can be used to test drug reactions on multiple organ systems and stand in for in vivo testing. Advanced biofabrication techniques of fiber assembly aim to address the challenge of creating physiologically realistic tissues through material and 3D mechanical structural manipulation. Artificial textile fibrous structures and their effect on the mechanical properties of engineered tissues is an underlying theme in this review. Fiber-based tissue engineered systems can be manufactured to mimic the mechanical properties of biological human tissue structures and further be seeded with cells, growth factors, and other biologics to provide suitable cellular compositions and tissue-induced signaling cues (7).
Current manufacturing methods such as electrospinning (8-10), wet spinning (11-13), microfluidic spinning (14-16), biospinning (17,18), interfacial complexation (19,20), melt spinning (21,22) produce fibers which are assembled through weaving, kitting, and braiding textile methods, to create precise compositions, geometries, and integrated structures. Electrospinning have been widely applied to biomedical tissue engineering for applications such as wound dressings and wound tissue substitutes (23); the inherent adaptability in fiber diameter, scaffold pore size, and material properties allows for a wide range of extra-cellular matrix (ECM) applications. Wet spinning methods can be used to fabricate fibers from bioactive polymers with porous structures and large pore size, to facilitate cell adhesion or encapsulation, proliferation, and migration (12). Used extensively in fiber-based tissue engineering application, biospinning of natural protein fibers results in high tensile strength and biocompatibility (17). Interfacial complexation allows for the encapsulation of cells and particles with several polyionic polymers (19,20). Meltspun fibers allow for various constructions which may facilitate improved cell alignment and orientation (21,22).
In this review, we will focus on applications in epithelial, connective, muscular, and nervous tissue engineering, where fiber-based microphysiological tissue constructs are utilized for the development of disease models and regenerative tissue engineering. These constructs have potential to be applied to high throughput drug screening as in vitro 3D models. Electrospinning, wet spinning, microfluidic spinning, biospinning, interfacial complexation, and melt spinning fabrication techniques for fibers with controllable mechanical properties, surface morphologies, and material compositions will be discussed. In addition, the advantages and limitations of warp and weft knitting, weaving, braiding, and winding textile methods of fiber assembly will be examined. The structural, physical, biological, and economical properties of the fabricated fibers are compared. Finally, emerging applications of fiber-based disease models and drug testing for research and clinical practice are explored.
Methods of fiber fabrication
Different approaches have been reported for the fabrication of micro and nanofibers from both natural and synthetic polymers. These fabrication techniques have significant effects on the fiber features such as the mechanical properties and size. Electrospinning, wet spinning, microfluidic spinning, mold casting, biospinning, interfacial complexation, and melt spinning are the main approaches for fiber fabrication and are shown in Figure 1. A description of each fabrication method is presented, and the benefits and drawbacks of each method are compared.
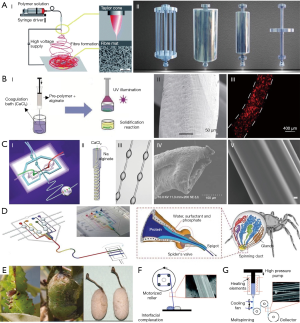
Electrospinning
During the electrospinning process, continuous fibers are formed by flowing a polymeric solution through needle by applying an electrical field between the needle and collector plate, placed a distance from the needle (30). The fiber diameter can be tuned from 100 nm to microns based on the physical properties of injected solution (i.e., concentration, viscosity, electrical conductivity), polymer solvent, solution flow rate, applied voltage, needle to collector distance, needle size, and the complexity of the collector (8). In addition, the crystallinity and orientation of the electrospun fibers are highly influenced by the type of collector. For instance, using stationary or rotating collectors enable the production of randomly oriented or aligned electrospun fibers, respectively (15,31). Numerous polymers such as collagen (9), gelatin (10), hyaluronic acid (32), silk fibroin (33), and chitosan (34) have been used to fabricate electrospun fibers for different biomedical applications. Electrospun mats and fibers have been used for manufacturing temporary tissue grafts (35), wound dressings, biosensors, and drug carriers (8); controlled, on-demand drug delivery can be achieved using thermally responsive electrospun synthetic and organic polymers (36,37). Electrospinning is a relatively simple method that enables precise control over the fabrication variables and allows for the possibility for production scale-up (38). However, this method brings with it significant challenges, like small pore size in electrospun fibers which limits cell penetration inside the electrospun mats, as well as poor ability to fabricate complex thick 3D structures (39,40). In addition, this method has a low potential for fabricating cell-laden fibers and encapsulating live cells, mainly due to the small fiber size, poor cell distribution within the polymeric solution, and cell exposure to potentially harmful solvents during the fabrication process (15,41).
Wet spinning
In wet spinning methods, a prepolymer solution (such as alginate) is extruded into a coagulation bath (such as Calcium Chloride 2%) in order to crosslink and form long continuous fibers (11). The injection of polymer can be carried out manually with a syringe pump (12) or through applied pressure (42). The polymeric solution is generally insoluble or poorly soluble in the coagulation solution. The size of wetspun fibers ranges from 30–600 µm and may be controlled by needle gauge, polymeric composition, and flow rate (15). Introducing various reinforcing components such as carbon nanotubes (43), calcium phosphates (44), and graphene oxide (45) to the polymer solution enhances the overall mechanical properties. Various structures including solid, hollow, and grooved fibers can be fabricated by wet spinning methods (46). In addition, this method can be extended to fabricate wetspun fibers from bioactive polymers such as gelatin, agarose, gelatin meth-acrylate (GelMA), poly (ethylene glycol) diacrylate (PEGDA), and poly (vinyl alcohol) (PVA) by entrapping the polymers into a sacrificial polymeric template like alginate (25), shown in Figure 1. Wetspun fibers can be randomly deposited onto a substrate or rolled up for scaffold fabrications (47,48). The fabricated fibers are relatively thick, with an intrinsic porous structure and large pore size which facilitates cell adhesion, proliferation, and migration into the fibers (12). Besides, cell laden fibers can be fabricated using this method by incorporating live cells in the initial polymer solution (13,49). However, depending on the application, long exposure to cytotoxic chemicals during the crosslinking process can limit the loading possibilities of cells within fibers (15).
Microfluidic spinning
The principles of microfluidic spinning are similar to that of wet spinning, such that fibers form inside microchannels by co-axial flows of prepolymer and crosslinking solutions. Coaxed streams of core and sheath solutions prevent crosslinking of fibers within the channels and leads to continuous fiber formation. These fibers may be rolled up using a rotating roller or fabricated into a scaffold with aligned fibers (15). The size of microfluidic spun fibers can be tuned by changing the solution flow rate, channel size, solution viscosity, and collecting roller rotation speed. The size of fabricated fibers may vary from a few to several hundreds of micrometers (50). Various structures such as grooved (51), hollow (26), helical (27), and spindle-knot (28) can be fabricated using microfluidic spinning to mimic biological structures. This method allows for the fabrication of heterotypic functional fibers in which compositional and topographical properties are varied by coding digital programable controls to mimic the biospun threads of spiders (3). Microfluidic spinning enables fabrication of cell-laden fibers and several studies have been conducted on this issue. For instance, Lee et al. created a microfluidic spun alginate fiber with a endothelial cell-loaded core structure, embedded within a smooth muscle cell-encapsulating bulk agar hydrogel (14). Shi et al. adopted microfluidic spinning techniques to fabricate GelMA grooved fiber, in which cells were simultaneously encapsulated within the fiber and seeded on the micro-grooved surface of the same fiber (16). Generally, this method has a high potential in fabricating biological fibers with various chemical compositions, morphological, and structural features. Recent advancements in microfluidic technology have enabled high-throughput production of microfluidic spun fibers (27,52-54). However, generally microfluidic spinning methods result in fibers with poor mechanical properties for use in typical textile scaffold fabrication methods.
Biospinning
Insects are used to fabricate silk fibers in biospinning fabrication. Silk is a natural protein fiber with high tensile strength and high biocompatibility, which has been extensively used in fiber-based tissue engineering applications. Silk can be derived from cocoons and nets created by insects such as worms and spiders, with a core of fibroin and a shell of sericin (17). The fibers can be obtained by natural or manual drawing from immobilized insects. The size of obtained fibers is a function of the method of drawing, such that the fiber diameters are 25–30 and 65–70 µm for manually and natural drawing, respectively (18). Since these fibers have relatively high tensile properties, they have been used for load bearing applications (17). However, this method is associated with major challenges such as resource limitations, lack of control over fabricated fiber diameter, lack of cell incorporation possibilities, procedure expense, and time required.
Interfacial complexation
In the interfacial complexation process, a solid fiber is fabricated from two oppositely charged polyelectrolyte droplets by drawing the interface upward using forceps or a bent needle. A mechanical roller can be used for drawing the fiber and for fabricating continuous fibers (19). Fiber size (10–20 µm) and tensile strength (20–200 MPa) are highly affected by the type and concentration of the polyelectrolytes, as well as interface area (15). Several polyionic polymers such as alginate, chitosan, and dextran sulphate have been used to fabricate fibers with this method (19). Interfacial complexation is simple method capable of encapsulating cells and particles as reported by Yim et al. (20). However, this method is not promising for industrial scale systems, as it is limited to few materials and small-scale fabrication. In addition, fabricating cell-laden structures has difficulties due to the limited size range of fibers.
Melt spinning
This process refers to making continuous fibers by heating a synthetic polymer like poly (3-hydroxybutyrate) to its melting point and extruding the molten polymer through a spinneret (21). Meltspun fibers can have various constructions such as multifilament and complex cross-sectional fibers, which may facilitate cell alignment and orientation (21,22). However, the high temperature requirement restricts the application to non-cell laden and protein loaded fibers. This method is also associated with other limitations such as expensive equipment, high pressure requirements, and technical issues during fabrication such as viscosity decrease and mass loss during fabrication (15,55,56).
Methods of fiber assembly
Once fibers have been fabricated, there are four main techniques of assembly to arrange them into useful 3D constructs. These are knitting, weaving, braiding, and winding; traditional textile techniques that are recently finding application in tissue engineering (57,58).
Knitting
Knitting is a well-known method of fiber assembly, where loops of materials are interlocked into complex structures. This technique can be used to produce 2D and 3D arrangements. One key advantage of the knitting process, especially in light of the precise nature of tissue engineering and drug screening, is the high formability of knitting (59). However, in the assembly process there is a difficulty associated with incorporating stiff, fragile or brittle fibers, as knit structures are inherently complex (60).
The process of knitting creates a structure by drawing fibers through pre-existing loops to form new, interconnected loops, as illustrated in Figure 2. The direction of the loops categorizes the knitting process into one of two subcategories, as either weft or warp knitting (59). Within these two broader subcategories exist a plethora of possible knitting structures, but only a limited number of these are considered practical for tissue engineering applications, due to considerations of strength and fabrication intricacies. Knitting can provide support both parallel to and normal to the fabric, thus allowing for the creation of 3D bio-structures (15). The characteristics of knitted structures are typically anisotropic and are dictated by the fibers and parameters of the knit, like stitch density (59). Coupled with fine control over the microstructure of knit structure granted by computer aided design systems, this means there is great potential for engineering highly specified tissue structures based on knitting (64). Knit constructs are very well defined—there exist mathematical models, originally defined by Leaf and Glaskin, for quantification of knit structures, based on parameters such as yarn diameter and loops per unit length (60). This model has been expanded upon by Ramakrishna et al. to develop a model for predicting the mechanical properties of a knit structure (65).
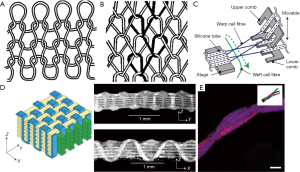
Weft knitting
A textile is considered to be weft-knitted if it is made from a single fiber, fed through the loops perpendicular to the direction that the textile is growing (60). An example of weft knitting is shown in Figure 2. Weft knitting is typically less stable than warp knitting, takes longer to produce, but is a simpler structure to assemble (59).
Warp knitting
By contrast, warp knitting uses a separate fiber for each needle, and forms loops that run lengthwise, parallel to the extrusion of the textile (60). The structure of warp knitting is shown below, in Figure 2. Warp knitting works by interlocking the loops into adjacent columns, creating a much more stable structure than weft knitting at potentially higher speeds, at the cost of increased manufacturing complexity (59).
Weaving
Weaving is a second technique for fiber assembly which relies on the use of a loom. In this process, a series of fibers are pre-stretched across the loom, called warp threads, and a weft thread is woven between them. The characteristics of the final textile can be tuned by adjusting the weaving parameters, such as the spacing or tension on the warp and weft threads (57). Typical looms are large affairs, designed to produce textiles for large-scale applications, but micro-sized looms have been built for microfluidic weaving, as shown in Figure 2. One example developed by Onoe et al. uses combs with affixed small hollow silicon tubes to guide the warp fibers, while the weft fiber was guided between them. The entire procedure was carried out in a liquid environment consisting of culture medium or PBS with calcium ions, which is important for hydrogel formation and cell culture (4). Akbari et al. similarly modified an off-the-shelf loom to work in a wet environment, for weaving hydrogel-coated fibers. This apparatus produced a woven structure that was mechanically sound, yet easily manipulated both during and after weaving (57). While weaving is typically a strictly 2D process (15), Moutos et al. developed a technique to create 3D woven structures to produce hydrogel composites for cell culture (63). This was accomplished by using a third set of fibers in the Z-direction to interlock the weft and warp fibers of the structure, as shown in Figure 2.
Braiding
Braiding is a simple textile technique, producing a more complex fiber from three or more fibers, and is suitable for producing complex 1D structures (64). Braiding has a number of possible applications, as it can be used to replicate physical characteristics of a structure, or to co-culture different loaded fibers together (57), as shown in Figure 2. In this example, Akbari et al. co-cultured human umbilical vein endothelial cells (HUVECs), fibroblasts, and hepatocytes by loading three different fibers with the respective cell types, and then braiding them to form a model of a liver (57). Braided fibers have found many applications, including tendon implants, orthopedic implants, and nerve guides (66).
Winding
The simplest fiber assembly technique in common use is winding. Winding around a mandrel is a typical storage technique for fibers but is finding some application in tissue engineering. For example, Akbari et al. wound cell-seeded fibers around a syringe needle to produce a hollow structure, potentially useful for producing blood vessels or similar physiological bodies (57). Altman et al. developed an engineered anterior cruciate ligament (ACL) replacement, using a matrix of twisted silk fibroin. The matrix produced was comprised of six parallel cords, each made up themselves of three strands wound counter clockwise together, each strand being comprised of six clockwise wound bundles of parallel fibers. The winding was performed with a winding machine. This complex helical structure provided an environment with multi-directional fibers similar to that of a native ACL, thus providing a suitable environment for cells to develop on, and closely mimicking the structural characteristics of the native tissue (67).
Disease model applications
The introduction of fiber-based textile techniques for fabricating complex tissue constructs has opened new avenues for engineering tissue and disease models with physiologically realistic mechanical and cellular properties. These tissue constructs suggest future improvements in drug screening applications. Fiber-based bioengineering and textile assembly approaches have been applied to a variety of tissue engineering and epithelial wound healing applications. Less complex methods of bulk 3D matrix culture exist for producing 3D tissue constructs, as demonstrated in several studies (68-70), however, these methods lack the mechanical property and material variability required for replicating the in vivo intricacies of biological tissues. Control over cell and biomolecule placement, material distribution, and construct geometry are all necessary for engineering structures with anisotropic characteristics like connective tissues bone (71) and tendon (72,73), and promoting unidirectional cell alignment in skeletal muscles (74), cardiac muscle tissue (75), and nervous tissue (76). Advanced fabrication methods can be utilized to create multi-layer composite fibers (4) and micropatterned fiber surface morphologies (74), through the manipulation of synthetic and organic biomaterial properties. This section outlines recent advancements and emerging applications in fiber fabrication and textile assemblies for epithelial, nervous, connective, and muscle tissue engineering for the purpose of disease modelling and therapeutic testing.
Epithelial tissue diseases
Basement membranes in kidney tubules are specialized extracellular membranes located beneath the epithelial cells, which provide necessary structural and tissue function support via growth factor signal regulation (77). Mollet et al. created a renal tubular basement membrane with ureidopyrimidinone-functionalized polymer and bioactive peptides using melt spinning, for use in a renal tissue model, shown in Figure 3. The basement membrane had biophysical properties like those of collagen IV and laminin. A monolayer of human kidney-2 epithelial cells demonstrate attachment, proliferation, and infiltration on the ECM and demonstrate modulation of gene expression and membrane transport proteins (78). The fabricated basement matrix acts an in vitro replacement of the natural ECM to support the growth of renal epithelial cells (78). Onoe et al. demonstrate the application of a microfluidic spinning device for creating double-coaxial meter-long hydrogel microfibers, with encapsulated ECM proteins, differentiated epithelial cells, and somatic stem cells. These fibers shown in Figure 3, are assembled by textile weaving and reeling techniques to mimic the intrinsic morphologies and functions of living epithelial tissues, and were shown to provide suitable microenvironments for cell behaviour in vivo (4). Artificial skin substitutes also offer effective therapeutic options for the treatment of burn wounds and chronic diabetic ulcers. Sheikh et al. demonstrated a cold-plate electrospinning technique with silk fibroin polymer for producing full-thickness 3D nanofiber skin scaffolds with high porosity and controllable sheet thickness (79). This method overcame prior difficulties involving poor cell infiltration into electrospun extracellular matrices due to uncontrolled fiber deposition, and leads to improved cell infiltration and water-binding abilities (79).
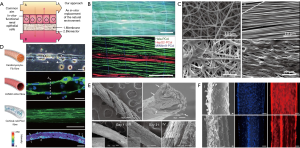
Nervous tissue diseases
Neurological disease phenotypes are presented through measurable changes in cell morphology such as neuronal process length and soma size (5). Nerve tissue engineering processes can be used to create physiologically accurate disease models for drug testing applications via the quantification of physical neuronal changes. Successful engineered neural tissues rely on controlled cellular behaviour and tissue progression by development of supporting scaffolds for cell cultures, akin to that of native ECM (80). Zamani et al. developed a synthetic scaffold environment by electrospinning onto a rotating drum collector, to support neurite outgrowth in specific orientations, with the purpose of bridging the gap across nerve defects through axon growth (76). Electrospun fiber scaffolds best influence neural cell growth when the fibres produced are aligned, as shown by the morphologies of A-172 neuron cells on scaffolds with different alignment geometries in Figure 3. The biocompatible, immunological inertness, and electrically conductive properties of the nanofiber electrospun scaffold promoted cell direction, morphology, and proliferation (76). Further work by Onoe et al. demonstrate the ability to encapsulate cortical cells and ECM proteins within hydrogel fibers, using a microfluidic spinning fabrication process. Neurons extended along the direction of the fiber forming a neuronal network (Figure 3) with spontaneous and synchronized Ca2+ oscillations. This suggests that the fiber environment exhibits the intrinsic functions required for regulating cellular interactions (4).
Connective tissue diseases
Current therapies for connective tissue repair generally result in poor clinical outcomes, due to the low regenerative capacity of tendons, inherently low cellular counts, and hypovascularity (73). Regenerative connective tissue constructs can improve these outcomes by incorporating cultured cells on scaffolds with structural and mechanical properties tunable to that of soft tissues, such as tendon and ligaments, and the connection points between soft tissues and bone. Costa-Almeida et al. have developed a composite suture fiber with a biodegradable cell-laden hydrogel layer for surgically repairing damaged tendons and anchoring tissue grafts. Human tendon-derived cells (hTDCs) were shown to migrate from the hydrogel layer and align along the core fiber direction. The collagen matrix produced by the hTDCs remodelled the environment to resemble native tendon tissue, shown in Figure 3. These fibers were also used in 3D constructs through textile processes such as braiding, to mimic the architecture, tensile strength, and load bearing capabilities of tendons and ligaments (73). A biodegradable scaffold for regeneration of the tendon-bone junction was developed by Ramakrishna et al., using textile braiding assembly methods to create tubular scaffolds from PLA yarns, cultured with joint progenitor cells (72). The tubular scaffolds for tendon and bone constructs were created with fibers of different surface morphologies. Cell viability, attachment, and proliferation was confirmed on all scaffolds, suggesting the potential use in regenerative surgical repairs. Further, the overall strength and elastic Young’s modulus of the scaffolds closely mimicked that of the separate tendon and bone components (72).
Muscle tissue diseases
Engineered functional muscle constructs have important applications in regenerative medicine for treating various debilitating muscular disorders through tissue repair and myofiber regeneration (81). Additionally, these muscle tissue models can be used to examine altered contractile responses to drugs and therapeutics. Bioengineered muscle tissues are generally been lacking in contractile properties comparable to native muscle tissues (74). Ebrahimi et al. developed a microfluidic spinning system for fabricating hydrogel fibers and examined the effects of topographical surface cues and biochemical stimulation on cultured C2C12 myoblasts. Micropatterned and agrin stimulated fibers promoted myoblast cell alignment and myotube formation during differentiation, thus improving the contractile function of engineered skeletal muscle tissues, shown in the contraction analysis in Figure 3. A wet spinning method for fabricating coaxial smooth-muscle tissue constructs was used by Hsiao et al. to yield self-assembling spring-like fibers with precise 3D orientation and alignment of encapsulated multipotent de-differentiated fat (DFAT) cells (82). These manufactured fibers mimicked the circumferential spatial organization of smooth muscle fibers located in many organs of the gastrointestinal, urinary, cardiovascular, and reproductive system, and showed differentiation of DFAT cells to smooth muscle lineage (82). These smooth muscle constructs show promise in regeneration of treating smooth muscles in vivo. Fleischer et al. developed a nanocomposite scaffold with gold nanoparticles and cardiac cells for heart muscle tissue (75). A static electrospinning method was used to fabricate coiled fiber scaffolds, to mimic the perimysium bundles of coiled fibers in native heart tissue, which yields its unique mechanical properties for continuous muscular contraction. The incorporation of gold nanoparticles ensured anisotropic electrical signal transfer between cardiac cells, allowing for higher contraction and relaxation forces of the engineered tissue, and the formation of elongated and aligned cardiac cells in vivo (75). Fiber-based engineered muscle tissue mimics native muscle and 3D constructs have the possibility of providing information on contractile muscle function in response to applied drugs and therapeutics.
Conclusions and future outlook
The development of fibrillar biofabrication methods in conjunction with assembly techniques akin to those used in textile applications has resulted in exciting research developments in the areas of tissue engineering, disease modelling, and drug screening. Each of the biofabrication techniques presented in this review offer different advantages and disadvantages for engineering complex tissue structures. Electrospinning is a relatively simple method which allows for production scale-up and precise control over variables. However, small scaffold pore size may limit cell penetration, and there are inherent limits to electrospun mat thickness for use as 3D structures. Wet spinning results in porous fibers that facilitate cell adhesion, proliferation, and inner migration, and also allows for the incorporation of live cells in the initial polymer solution. However, long exposure to cytotoxic chemicals in the crosslinking process can limit the loading possibilities of cells within fibers. Microfluidic spinning is used to fabricate fibers with a large range of chemical compositions, morphological, and structural features. The resulting fibers have relatively poor mechanical properties for use with typical textile scaffold fabrication methods, however.
The different fiber assembly methods presented again offer advantages and disadvantages for engineered tissue constructs, depending on the required structural morphologies. Knitting provides both parallel and perpendicular support, which allows for the creation of 3D structures with fine control over the microstructure of highly specified tissue structures. However, this inherent complexity makes incorporating stiff, fragile or brittle fibers more difficult. While weaving commonly produces only 2D structures, these structures can be converted into 3D, which can be taken advantage of for tissue scaffold and cell culture applications. Braiding is a very simple textile technique but is only suitable for producing complex 1D structures. Winding is the simplest fiber assembly technique and can produce multi-directional helical structures that mimic a variety of physiological tissue constructs such as ligaments and circumferential smooth muscle fibers located in many organs of the gastrointestinal, urinary, cardiovascular, and reproductive system.
The combination of biofabrication and assembly techniques has enabled the fabrication of complex fiber-based microphysiological tissue systems. These techniques have also provided an effective means of employing careful control over cell placement, surface and construct geometry for engineering structures with anisotropic characteristics like connective tissues bone (71) and tendon (72,73), as well as promoting unidirectional cell alignment in skeletal muscles (74), cardiac muscle tissue (75), and nerves (76). Advanced fabrication methods have been utilized to create multi-layer composite fibers (4) and electrospun full-thickness 3D nanofiber skin scaffolds with high cellular infiltration (79), to mimic the intrinsic morphologies and functions of living epithelial tissues through the manipulation of synthetic and organic biomaterial properties.
Overall, these advances in biofabrication techniques of bio-fibers and constructs, hold great promise in fields of regenerative tissue engineering, disease modelling, and suggest future improvements in platforms for high throughput testing of chemical compounds, drugs, and therapeutics.
Acknowledgments
Funding: M Akbari and H Dabiri acknowledge the funding received from the Natural Sciences and Engineering Research Council of Canada (NSERC) discovery grant. M Akbari, T Walsh, and L Karperien acknowledge the funding received from the Canadian Institutes for Health Researches (CIHR). H Dabiri also thanks RepliCel Life Sciences for their financial support.
Footnote
Conflicts of Interest: All authors have completed the ICMJE uniform disclosure form (available at http://dx.doi.org/10.21037/mps.2019.08.01). MA serves as an unpaid editorial board member of Microphysiological Systems from Feb 2017 to Jan 2020. The other authors have no conflicts of interest to declare.
Ethical Statement: The authors are accountable for all aspects of the work in ensuring that questions related to the accuracy or integrity of any part of the work are appropriately investigated and resolved.
Open Access Statement: This is an Open Access article distributed in accordance with the Creative Commons Attribution-NonCommercial-NoDerivs 4.0 International License (CC BY-NC-ND 4.0), which permits the non-commercial replication and distribution of the article with the strict proviso that no changes or edits are made and the original work is properly cited (including links to both the formal publication through the relevant DOI and the license). See: https://creativecommons.org/licenses/by-nc-nd/4.0/.
References
- Szymański P, Markowicz M, Mikiciuk-Olasik E. Adaptation of high-throughput screening in drug discovery-toxicological screening tests. Int J Mol Sci 2012;13:427-52. [Crossref] [PubMed]
- De Witte TM, Fratila-Apachitei LE, Zadpoor AA, et al. Bone tissue engineering via growth factor delivery: from scaffolds to complex matrices. Regen Biomater 2018;5:197-211. [Crossref] [PubMed]
- Kang E, Jeong GS, Choi YY, et al. Digitally tunable physicochemical coding of material composition and topography in continuous microfibres. Nat Mater 2011;10:877-83. [Crossref] [PubMed]
- Onoe H, Okitsu T, Itou A, et al. Metre-long cell-laden microfibres exhibit tissue morphologies and functions. Nat Mater 2013;12:584-90. [Crossref] [PubMed]
- Xu XH, Zhong Z. Disease modeling and drug screening for neurological diseases using human induced pluripotent stem cells. Acta Pharmacol Sin 2013;34:755-64. [Crossref] [PubMed]
- Broach JR, Thorner J. High-throughput screening for drug discovery. Nature 1996;384:14-6. [PubMed]
- Langer R, Vacanti JP. Tissue Engineering. Science 1993;260:920-6. [PubMed]
- Mirjalili M, Zohoori S. Review for application of electrospinning and electrospun nanofibers technology in textile industry. J Nanostructure Chem 2016;6:207-13. [Crossref]
- Rho KS, Jeong L, Lee G, et al. Electrospinning of collagen nanofibers: Effects on the behavior of normal human keratinocytes and early-stage wound healing. Biomaterials 2006;27:1452-61. [Crossref] [PubMed]
- Topuz F, Uyar T. Electrospinning of gelatin with tunable fiber morphology from round to flat/ribbon. Mater Sci Eng C Mater Biol Appl 2017;80:371-8. [Crossref] [PubMed]
- Hu X, Rajendran S, Yao Y, et al. A novel wet-spinning method of manufacturing continuous bio-inspired composites based on graphene oxide and sodium alginate. Nano Res 2016;9:735-44. [Crossref]
- Puppi D, Dinucci D, Bartoli C, et al. Development of 3D wet-spun polymeric scaffolds loaded with antimicrobial agents for bone engineering. J Bioact Compat Pol 2011;26:478-92. [Crossref]
- Arumuganathar S, Jayasinghe SN. Living scaffolds (Specialized and unspecialized) for regenerative and therapeutic medicine. Biomacromolecules 2008;9:759-66. [Crossref] [PubMed]
- Lee KH, Shin SJ, Park Y, et al. Synthesis of Cell-Laden Alginate Hollow Fibers Using Microfluidic Chips and Microvascularized Tissue-Engineering Applications. Small 2009;5:1264-8. [Crossref] [PubMed]
- Tamayol A, Akbari M, Annabi N, et al. Fiber-based tissue engineering: Progress, challenges, and opportunities. Biotechnol Adv 2013;31:669-87. [Crossref] [PubMed]
- Shi X, Ostrovidov S, Zhao Y, et al. Microfluidic Spinning of Cell-Responsive Grooved Microfibers. Adv Funct Mater 2015;25:2250-9. [Crossref]
- Mottaghitalab F, Hosseinkhani H, Shokrgozar MA, et al. Silk as a potential candidate for bone tissue engineering. J Control Release 2015;215:112-28. [Crossref] [PubMed]
- Mandal BB, Kundu SC. Biospinning by silkworms: Silk fiber matrices for tissue engineering applications. Acta Biomaterialia 2010;6:360-71. [Crossref] [PubMed]
- Wan ACA, Liao IC, Yim EKF, et al. Mechanism of fiber formation by interfacial polyelectrolyte complexation. Macromolecules 2004;37:7019-25. [Crossref]
- Yim EKF, Wan ACA, Le Visage C, et al. Proliferation and differentiation of human mesenchymal stem cell encapsulated in polyelectrolyte complexation fibrous scaffold. Biomaterials 2006;27:6111-22. [Crossref] [PubMed]
- Hinuber C, Haussler L, Vogel R, et al. Hollow Poly(3-hydroxybutyrate) Fibers Produced by Melt Spinning. Macromol Mater Eng 2010;295:585-94. [Crossref]
- Ellä V, Annala T, Länsman S, et al. Knitted polylactide 96/4 L/D structures and scaffolds for tissue engineering: shelf life, in vitro and in vivo studies. Biomatter 2011;1:102-13. [Crossref] [PubMed]
- Li Y, Bou-Akl T. Electrospinning in Tissue Engineering. Electrospinning - Material, Techniques and Biomedical Applications. IntechOpen, 2016:117-39.
- Wallace GG, Higgins MJ, Moulton SE, et al. Nanobionics: the impact of nanotechnology on implantable medical bionic devices. Nanoscale 2012;4:4327-47. [Crossref] [PubMed]
- Tamayol A, Najafabadi AH, Aliakbarian B, et al. Hydrogel Templates for Rapid Manufacturing of Bioactive Fibers and 3D Constructs. Adv Healthc Mater 2015;4:2146-53. [Crossref] [PubMed]
- Yu Y, Wen H, Ma J, et al. Flexible fabrication of biomimetic bamboo-like hybrid microfibers. Adv Mater 2014;26:2494-9. [Crossref] [PubMed]
- Xu P, Xie R, Liu Y, et al. Bioinspired Microfibers with Embedded Perfusable Helical Channels. Adv Mater. 2017; [Crossref] [PubMed]
- Shang L, Fu F, Cheng Y, et al. Bioinspired Multifunctional Spindle-Knotted Microfibers from Microfluidics. Small 2017; [Crossref] [PubMed]
- Kundu SC, Kundu B, Talukdar S, et al. Invited review nonmulberry silk biopolymers. Biopolymers 2012;97:455-67. [Crossref] [PubMed]
- Pillay V, Dott C, Choonara YE, et al. A Review of the Effect of Processing Variables on the Fabrication of Electrospun Nanofibers for Drug Delivery Applications. J Nanomater 2013; [Crossref]
- Kim KW, Lee KH, Khil MS, et al. The effect of molecular weight and the linear velocity of drum surface on the properties of electrospun poly(ethylene terephthalate) nonwovens. Fiber Polym 2004;5:122-7. [Crossref]
- Chen CH, Chen SH, Shalumon KT, et al. Dual functional core-sheath electrospun hyaluronic acid/polycaprolactone nanofibrous membranes embedded with silver nanoparticles for prevention of peritendinous adhesion. Acta Biomaterialia 2015;26:225-35. [Crossref] [PubMed]
- Melke J, Midha S, Ghosh S, et al. Silk fibroin as biomaterial for bone tissue engineering. Acta Biomaterialia 2016;31:1-16. [Crossref] [PubMed]
- Kohsari I, Shariatinia Z, Pourmortazavi SM. Antibacterial electrospun chitosan-polyethylene oxide nanocomposite mats containing ZIF-8 nanoparticles. International Journal of Biological Macromolecules 2016;91:778-88. [Crossref] [PubMed]
- Mahmoudi N, Eslahi N, Mehdipour A, et al. Temporary skin grafts based on hybrid graphene oxide-natural biopolymer nanofibers as effective wound healing substitutes: pre-clinical and pathological studies in animal models. J Mater Sci Mater Med 2017;28:73. [Crossref] [PubMed]
- Tamayol A, Najafabadi AH, Mostafalu P, et al. Biodegradable elastic nanofibrous platforms with integrated flexible heaters for on-demand drug delivery. Sci Rep 2017;7:9220. [Crossref] [PubMed]
- Mohammadi S, Ramakrishna S, Laurent S, et al. Fabrication of Nanofibrous PVA/Alginate-Sulfate Substrates for Growth Factor Delivery. J Biomed Mater Res A 2019;107:403-13. [Crossref] [PubMed]
- Deng M, James R, Laurencin CT, et al. Nanostructured polymeric scaffolds for orthopaedic regenerative engineering. IEEE Trans Nanobioscience 2012;11:3-14. [Crossref] [PubMed]
- Shabani I, Haddadi-Asl V, Seyedjafari E, et al. Cellular infiltration on nanofibrous scaffolds using a modified electrospinning technique. Biochem Biophys Res Commun 2012;423:50-4. [Crossref] [PubMed]
- Cheng J, Jun Y, Qin JH, et al. Electrospinning versus microfluidic spinning of functional fibers for biomedical applications. Biomaterials 2017;114:121-43. [Crossref] [PubMed]
- López-Rubio A, Sanchez E, Sanz Y, et al. Encapsulation of Living Bifidobacteria in Ultrathin PVOH Electrospun Fibers. Biomacromolecules 2009;10:2823-9. [Crossref] [PubMed]
- Jayasinghe SN, Suter N. Pressure driven spinning: A multifaceted approach for preparing nanoscaled functionalized fibers, scaffolds, and membranes with advanced materials. Biomicrofluidics 2010;4:14106. [Crossref] [PubMed]
- Jestin S, Poulin P. Wet Spinning of CNT-based Fibers. In: Schulz M, Shanov V, Yin Z. editors. Nanotube Superfiber Materials: Changing Engineering Design. William Andrew, 2013:167-209.
- Reddy N, Yang Y. Additives to Improve Performance of Alginate Fiber. In: Innovative Biofibers from Renewable Resources. Springer, 2014:139-45.
- Ji XQ, Xu YH, Zhang WL, et al. Review of functionalization, structure and properties of graphene/polymer composite fibers. COMPOS PART A-APPL S 2016;87:29-45. [Crossref]
- Yang Y, Sun J, Liu XL, et al. Wet-spinning fabrication of shear-patterned alginate hydrogel microfibers and the guidance of cell alignment. Regenerative Biomaterials 2017;4:299-307. [Crossref] [PubMed]
- Leonor IB, Rodrigues GT, Gomes ME, et al. In situ functionalization of wet-spun fibre meshes for bone tissue engineering. J Tissue Eng Regen Med 2011;5:104-11. [Crossref] [PubMed]
- Landers R, Pfister A, Hubner U, et al. Fabrication of soft tissue engineering scaffolds by means of rapid prototyping techniques. J Mater Sci 2002;37:3107-16. [Crossref]
- Arumuganathar S, Irvine S, McEwan JR, et al. A novel direct aerodynamically assisted threading methodology for generating biologically viable microthreads encapsulating living primary cells. J Appl Polym Sci 2008;107:1215-25. [Crossref]
- Jun Y, Kang E, Chae S, et al. Microfluidic spinning of micro- and nano-scale fibers for tissue engineering. Lab Chip 2014;14:2145-60. [Crossref] [PubMed]
- Kang E, Choi YY, Chae SK, et al. Microfluidic Spinning of Flat Alginate Fibers with Grooves for Cell-Aligning Scaffolds. Adv Mater 2012;24:4271-7. [Crossref] [PubMed]
- Cho S, Shim TS, Yang SM. High-throughput optofluidic platforms for mosaicked microfibers toward multiplex analysis of biomolecules. Lab Chip 2012;12:3676-9. [Crossref] [PubMed]
- Mirani B, Pagan E, Shojaei S, et al. A 3D bioprinted hydrogel mesh loaded with all-trans retinoic acid for treatment of glioblastoma. Eur J Pharmacol 2019;854:201-12. [Crossref] [PubMed]
- Mirani B, Pagan E, Currie B, et al. An Advanced Multifunctional Hydrogel-Based Dressing for Wound Monitoring and Drug Delivery. Adv Healthc Mater 2017;6:1700718 [Crossref] [PubMed]
- Akbari M, Sinton D, Bahrami M. Viscous flow in variable cross-section microchannels of arbitrary shapes. Int J Heat Mass Tran 2011;54:3970-8. [Crossref]
- Fakirov S, Bhattacharyya D. Handbook of engineering biopolymers : homopolymers, blends, and composites: Carl Hanser Verlag. GmbH & Co. KG, 2007.
- Akbari M, Tamayol A, Laforte V, et al. Composite Living Fibers for Creating Tissue Constructs Using Textile Techniques. Adv Funct Mater 2014;24:4060-7. [Crossref] [PubMed]
- Pedde RD, Mirani B, Navaei A, et al. Emerging Biofabrication Strategies for Engineering Complex Tissue Constructs. Adv Mater 2017;29:1606061 [Crossref] [PubMed]
- Leong KH, Ramakrishna S, Huang ZM, et al. Potential of knitting for engineering composites - a review. COMPOS PART A-APPL S 2000;31:197-220. [Crossref]
- Huang ZM, Ramakrishna S. Micromechanical modeling approaches for the stiffness and strength of knitted fabric composites: a review and comparative study. COMPOS PART A-APPL S 2000;31:479-501. [Crossref]
- Sagona C. Two-needle knitting and cross-knit looping: early bronze age pottery imprints from anatolia and the caucasus. Oxford J Archaeol 2018;37:283-97. [Crossref]
- Rozant O, Michaud V, Bourban PE, et al. A model for the consolidation of warp-knitted reinforced laminates. Polym Composite 2001;22:432-43. [Crossref]
- Moutos FT, Freed LE, Guilak F. A biomimetic three-dimensional woven composite scaffold for functional tissue engineering of cartilage. Nat Mater 2007;6:162-7. [Crossref] [PubMed]
- Akbari M, Tamayol A, Bagherifard S, et al. Textile Technologies and Tissue Engineering: A Path Toward Organ Weaving. Adv Healthc Mater 2016;5:751-66. [Crossref] [PubMed]
- Ramakrishna S, Huang ZM, Teoh SH, et al. Application of the model of leaf and glaskin to estimating the 3D elastic properties of knitted-fabric-reinforced composites. J Text I 2000;91:132-50. [Crossref]
- Li G, Li Y, Chen GQ, et al. Silk-Based Biomaterials in Biomedical Textiles and Fiber-Based Implants. Adv Healthc Mater 2015;4:1134-51. [Crossref] [PubMed]
- Altman GH, Horan RL, Lu HH, et al. Silk matrix for tissue engineered anterior cruciate ligaments. Biomaterials 2002;23:4131-41. [Crossref] [PubMed]
- Yodmuang S, McNamara SL, Nover AB, et al. Silk microfiber-reinforced silk hydrogel composites for functional cartilage tissue repair. Acta Biomaterialia 2015;11:27-36. [Crossref] [PubMed]
- Wang Y, Kim UJ, Blasioli DJ, et al. In vitro cartilage tissue engineering with 3D porous aqueous-derived silk scaffolds and mesenchymal stem cells. Biomaterials 2005;26:7082-94. [Crossref] [PubMed]
- Yuan D, Somers SM, Grayson WL, et al. A Poroelastic Model of a Fibrous-Porous Tissue Engineering Scaffold. Sci Rep 2018;8:5043. [Crossref] [PubMed]
- Salifu AA, Lekakou C, Labeed FH. Electrospun oriented gelatin-hydroxyapatite fiber scaffolds for bone tissue engineering. J Biomed Mater Res A 2017;105:1911-26. [Crossref] [PubMed]
- Ramakrishna H, Li T, He T, et al. Tissue engineering a tendon-bone junction with biodegradable braided scaffolds. Biomater Res 2019;23:11. [Crossref] [PubMed]
- Costa-Almeida R, Domingues RMA, Fallahi A, et al. Cell-laden composite suture threads for repairing damaged tendons. J Tissue Eng Regen Med 2018;12:1039-48. [Crossref] [PubMed]
- Ebrahimi M, Ostrovidov S, Salehi S, et al. Enhanced skeletal muscle formation on microfluidic spun gelatin methacryloyl (GelMA) fibres using surface patterning and agrin treatment. J Tissue Eng Regen Med 2018;12:2151-63. [Crossref] [PubMed]
- Fleischer S, Shevach M, Feiner R, et al. Coiled Fiber Scaffolds Embedded With Gold Nanoparticles Improve The Performance Of Engineered Cardiac Tissues. Nanoscale 2014;6:9410-4. [Crossref] [PubMed]
- Zamani F, Latifi M, Amani-Tehran M, et al. Effects of PLGA nanofibrous scaffolds structure on nerve cell directional proliferation and morphology. Fiber Polym 2013;14:698-702. [Crossref]
- Yurchenco PD. Basement Membranes: Cell Scaffoldings and Signaling Platforms. Cold Spring Harb Perspect Biol 2011; [Crossref] [PubMed]
- Mollet BB, Bogaerts ILJ, van Almen GC, et al. A bioartificial environment for kidney epithelial cells based on a supramolecular polymer basement membrane mimic and an organotypical culture system. J Tissue Eng Regen Med 2017;11:1820-34. [Crossref] [PubMed]
- Sheikh FA, Ju HW, Lee JM, et al. 3D electrospun silk fibroin nanofibers for fabrication of artificial skin. Nanomedicine 2015;11:681-91. [Crossref] [PubMed]
- Subramanian A, Krishnan UM, Sethuraman S. Development of biomaterial scaffold for nerve tissue engineering: Biomaterial mediated neural regeneration. J Biomed Sci 2009;16:108. [Crossref] [PubMed]
- Nam KH, Smith AS, Lone S, et al. Biomimetic 3D Tissue Models for Advanced High-Throughput Drug Screening. J Lab Autom 2015;20:201-15. [Crossref] [PubMed]
- Hsiao AY, Okitsu T, Onoe H, et al. Smooth Muscle-Like Tissue Constructs with Circumferentially Oriented Cells Formed by the Cell Fiber Technology. Plos One 2015;10:e0119010 [Crossref] [PubMed]
Cite this article as: Walsh T, Karperien L, Dabiri SMH, Akbari M. Fiber-based microphysiological systems: a powerful tool for high throughput drug screening. Microphysiol Syst 2019;3:3.